Sulekha Rani.R ,PGT Chemistry,KV NTPC Kayamkulam
My self Sulekha Rani.R,P.G.T Chemistry,KV INS Dronacharya , Cochin, Kerala .
Search This Blog
Thursday, July 5, 2012
Higgs Boson...New Innovation... 4 July-2012
Sulekha Rani.R ,PGT Chemistry,KV NTPC Kayamkulam
Higgs within reach….
Our understanding of the universe is about to change…
(A proton-proton collision event in the CMS experiment
producing two high-energy photons (red towers). This is what we would expect to
see from the decay of a Higgs boson but it is also consistent with background
Standard Model physics processes. © CERN 2012)
The ATLAS and CMS experiments at CERN today presented their latest
results in the search for the long-sought Higgs
boson. Both experiments see strong
indications for the presence of a new particle, which could be the Higgs boson,
in the mass region around 126 gigaelectronvolts (GeV).
The experiments found hints of the new
particle by analysing trillions of proton-proton collisions from the Large Hadron Collider (LHC) in 2011 and 2012. TheStandard
Model of particle physics predicts that a
Higgs boson would decay into different particles – which the LHC experiments
then detect.
Both ATLAS and CMS
gave the level of significance of the result as 5 sigma on the scale that
particle physicists use to describe the certainty of a discovery. One sigma
means the results could be random fluctuations in the data, 3 sigma counts as
an observation and a 5-sigma result is a discovery. The results presented today
are preliminary, as the data from 2012 is still under analysis. The complete
analysis is expected to be published around the end of July.
Press Release….
CERN experiments
observe particle consistent with long-sought Higgs boson
Geneva, 4 July 2012. At a
seminar held at CERN today as a curtain raiser
to the year’s major particle physics conference, ICHEP2012 in Melbourne, the
ATLAS and CMS experiments presented their latest preliminary results in the
search for the long sought Higgs particle. Both experiments observe a new
particle in the mass region around 125-126 GeV.
“We
observe in our data clear signs of a new particle, at the level of 5 sigma, in
the mass region around 126 GeV. The outstanding performance of the LHC and
ATLAS and the huge efforts of many people have brought us to this exciting
stage,” said ATLAS experiment spokesperson Fabiola Gianotti, “but a little more time is needed to prepare
these results for publication.”
"The
results are preliminary but the 5 sigma signal at around 125 GeV we’re seeing
is dramatic. This is indeed a new particle. We know it must be a boson and it’s
the heaviest boson ever found,” said CMS experiment spokesperson Joe Incandela. “The implications are very significant and
it is precisely for this reason that we must be extremely diligent in all of
our studies and cross-checks."
“It’s
hard not to get excited by these results,” said CERN Research Director Sergio Bertolucci. “
We stated last year that in 2012 we would either find a new Higgs-like particle
or exclude the existence of the Standard Model Higgs. With all the necessary
caution, it looks to me that we are at a branching point: the observation of
this new particle indicates the path for the future towards a more detailed
understanding of what we’re seeing in the data.”
The results presented today
are labelled preliminary. They are based on data collected in 2011 and 2012,
with the 2012 data still under analysis. Publication of the analyses
shown today is expected around the end of July. A more complete picture of
today’s observations will emerge later this year after the LHC provides the
experiments with more data.
The next step will be to
determine the precise nature of the particle and its significance for our
understanding of the universe. Are its properties as expected for the
long-sought Higgs boson, the final missing ingredient in the Standard Model of
particle physics? Or is it something more exotic? The Standard Model describes
the fundamental particles from which we, and every visible thing in the
universe, are made, and the forces acting between them. All the matter that we
can see, however, appears to be no more than about 4% of the total. A more
exotic version of the Higgs particle could be a bridge to understanding the 96%
of the universe that remains obscure.
“We
have reached a milestone in our understanding of nature,” said CERN Director General
Rolf Heuer. “The discovery of a particle consistent
with the Higgs boson opens the way to more detailed studies, requiring larger
statistics, which will pin down the new particle’s properties, and is likely to
shed light on other mysteries of our universe.”
Positive identification of
the new particle’s characteristics will take considerable time and data. But
whatever form the Higgs particle takes, our knowledge of the fundamental
structure of matter is about to take a major step forward.
(((((About CERN…CERN, the
European Organization for Nuclear Research, is the world's leading laboratory
for particle physics. It has its headquarters in Geneva. At present, its Member
States are Austria, Belgium, Bulgaria, the Czech Republic, Denmark, Finland,
France, Germany, Greece, Hungary, Italy, the Netherlands, Norway, Poland,
Portugal, Slovakia, Spain, Sweden, Switzerland and the United Kingdom. Romania
is a candidate for accession. Israel and Serbia are Associate Members in the
pre-stage to Membership. India, Japan, the Russian Federation, the United
States of America, Turkey, the European Commission and UNESCO have Observer
status.)))))
The Large Hadron Collider.... LHC
The Large Hadron Collider --- LHC
Our understanding of the Universe is about to change...
Sulekha Rani R,PGT Chemistry,KV NTPC Kayamkulam
The Large Hadron Collider (LHC) is a gigantic scientific instrument near Geneva, where it spans the border between Switzerland and France about 100m underground. It is a particle accelerator used by physicists to study the smallest known particles – the fundamental building blocks of all things. It will revolutionise our understanding, from the minuscule world deep within atoms to the vastness of the Universe.
Two beams of subatomic particles called "hadrons" – either protons or lead ions – travel in opposite directions inside the circular accelerator, gaining energy with every lap. Physicists use the LHC to recreate the conditions just after the Big Bang, by colliding the two beams head-on at very high energy. Teams of physicists from around the world then analyse the particles created in the collisions using special detectors in a number of experiments dedicated to the LHC.
There are many theories as to what will result from these collisions. For decades, the Standard Model of particle physics has served physicists well as a means of understanding the fundamental laws of Nature, but it does not tell the whole story. Only experimental data using the high energies reached by the LHC can push knowledge forward, challenging those who seek confirmation of established knowledge, and those who dare to dream beyond the paradigm

How the LHC works
The LHC, the world’s largest and most powerful particle accelerator, is the latest addition to CERN’s accelerator complex. It mainly consists of a 27-kilometre ring of superconducting magnets with a number of accelerating structures to boost the energy of the particles along the way.
Inside the accelerator, two beams of particles travel at close to the speed of light with very high energies before colliding with one another. The beams travel in opposite directions in separate beam pipes – two tubes kept at ultrahigh vacuum. They are guided around the accelerator ring by a strong magnetic field, achieved using superconducting electromagnets. These are built from coils of special electric cable that operates in a superconducting state, efficiently conducting electricity without resistance or loss of energy. This requires chilling the magnets to about ‑271°C – a temperature colder than outer space. For this reason, much of the accelerator is connected to a distribution system of liquid helium, which cools the magnets, as well as to other supply services.
Thousands of magnets of different varieties and sizes are used to direct the beams around the accelerator. These include 1232 dipole magnets of 15m length which are used to bend the beams, and 392 quadrupole magnets, each 5–7m long, to focus the beams. Just prior to collision, another type of magnet is used to "squeeze" the particles closer together to increase the chances of collisions. The particles are so tiny that the task of making them collide is akin to firing needles from two positions 10km apart with such precision that they meet halfway!
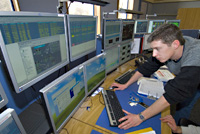
Model of an LHC superconducting dipole magnet

Heavy-ion... at the LHC
Preparation for injection in the LINAC 3 of the LEAD source material used to create heavy ions for the LHC.
In the LHC heavy-ion programme, beams of heavy nuclei ("ions") collide at energies up to 30 times higher than in previous laboratory experiments. In these heavy-ion collisions, matter is heated to more than 100,000 times the temperature at the centre of the Sun, reaching conditions that existed in the first microseconds after the Big Bang. The aim of the heavy-ion programme at the LHC is to produce this matter at the highest temperatures and densities ever studied in the laboratory, and to investigate its properties in detail. This is expected to lead to basic new insights into the nature of the strong interaction between fundamental particles.
The strong interaction is the fundamental force that binds Nature's elementary particles, called quarks, into bigger objects such as protons and neutrons, which are themselves the building blocks of the atomic elements. Much is known today about the mechanism with which the elementary force-carriers of the strong interaction, the gluons, bind quarks together into protons and neutrons. However, two aspects of the strong interaction remain particularly intriguing.
First, no quark has ever been observed in isolation: quarks and gluons seem to be confined permanently inside composite particles, such as protons and neutrons. Second, protons and neutrons contain three quarks, but the mass of these three quarks accounts for only one percent of the total mass of a proton or neutron. So while the Higgs mechanism could give rise to the masses of the individual quarks, it cannot account for most of the mass of ordinary matter.
The current theory of strong interactions, called quantum chromodynamics, predicts that at very high temperatures, quarks and gluons are deconfined and can exist freely in a new state of matter known as the quark-gluon plasma. Theory also predicts that at the same temperature, the mechanism that is responsible for giving composite particles most of their mass ceases to act.
In the LHC heavy-ion programme, three experiments – ALICE, ATLAS and CMS – aim to produce and study this extreme, high-temperature phase of matter and provide novel access to the question of how most of the mass of visible matter in the Universe was generated in the first microseconds after the Big Bang.
The LHC experiments
A worker inside the LHC tunnel
The six experiments at the LHC are all run by international collaborations, bringing together scientists from institutes all over the world. Each experiment is distinct, characterised by its unique particle detector.
The two large experiments, ATLAS and CMS, are based on general-purpose detectors to analyse the myriad of particles produced by the collisions in the accelerator. They are designed to investigate the largest range of physics possible. Having two independently designed detectors is vital for cross-confirmation of any new discoveries made.
Two medium-size experiments, ALICE and LHCb, have specialised detectors for analysing the LHC collisions in relation to specific phenomena.
Two further experiments, TOTEM and LHCf, are much smaller in size. They are designed to focus on "forward particles" (protons or heavy ions). These are particles that just brush past each other as the beams collide, rather than meeting head-on.
The ATLAS, CMS, ALICE and LHCb detectors are installed in four huge underground caverns located around the ring of the LHC. The detectors used by the TOTEM experiment are positioned near the CMS detector, whereas those used by LHCf are near the ATLAS detector.
What is Higgs boson
By Sulekha Rani.R ,PGT Chemistry , KV NTPC Kayamkulam
Higgs boson
The Standard Model successfully describes all of the elementary particles we know to exist and how they interact with one another. But our understanding of nature is incomplete. In particular, the Standard Model cannot answer one basic question: Why do most of these elementary particles have masses?
Without mass, the universe would be a very different place. For example, if the electron had no mass, there would be no atoms. Hence there would be no ordinary matter as we know it, no chemistry, no biology and no people. In addition, the Sun shines thanks to a delicate interplay among the fundamental forces of nature, which would be completely upset if some of those force particles did not have large masses.
At first sight the concept of mass seems not to fit into the Standard Model of particle physics. Two of the forces the model describes – electromagnetism and the weak nuclear force – can be described by a single theory, that of the electroweak force. Scientists have subjected the electroweak theory to many experimental tests, which it has passed with flying colours. However, the basic equations of the theory seem to require all elementary particles to be massless.
Scientists needed a way out of this conundrum. Several physicists, including Peter Higgs, discovered a mechanism that, if added to the equations, would allow particles to have masses. This is now known as the Higgs mechanism. Integrating it into the Standard Model allowed scientists to make predictions of various quantities, including the mass of the heaviest known particle, the top quark. Experimentalists found this particle just where equations using the Higgs mechanism predicted it should be.
According to theory, the Higgs mechanism works as a medium that exists everywhere in space. Particles gain mass by interacting with this medium. Peter Higgs pointed out that the mechanism required the existence of an unseen particle, which we now call the Higgs boson. The Higgs boson is the fundamental component of the Higgs medium, much as the photon is the fundamental component of light.
The Higgs boson is the only particle predicted by the Standard Model that has not yet been seen by experiments. The Higgs mechanism does not predict the mass of the Higgs boson itself but rather a range of masses. Fortunately, the Higgs boson would leave a unique particle footprint depending on its mass. So scientists know what to look for and would be able to calculate its mass from the particles they saw in the detector.
Experimentalists might find that the Higgs boson is different from the simplest version the Standard Model predicts. Many theories that describe physics beyond the Standard Model, such as supersymmetry and composite models, suggest the existence of a zoo of new particles, including different kinds of Higgs bosons. If any of these scenarios turn out to be true, finding the Higgs boson could be a gateway to discovering new physics, such as superparticles or dark matter. On the other hand, finding no Higgs boson at the LHC would give credence to another class of theories that explain the Higgs mechanism in different ways.
Subscribe to:
Posts (Atom)